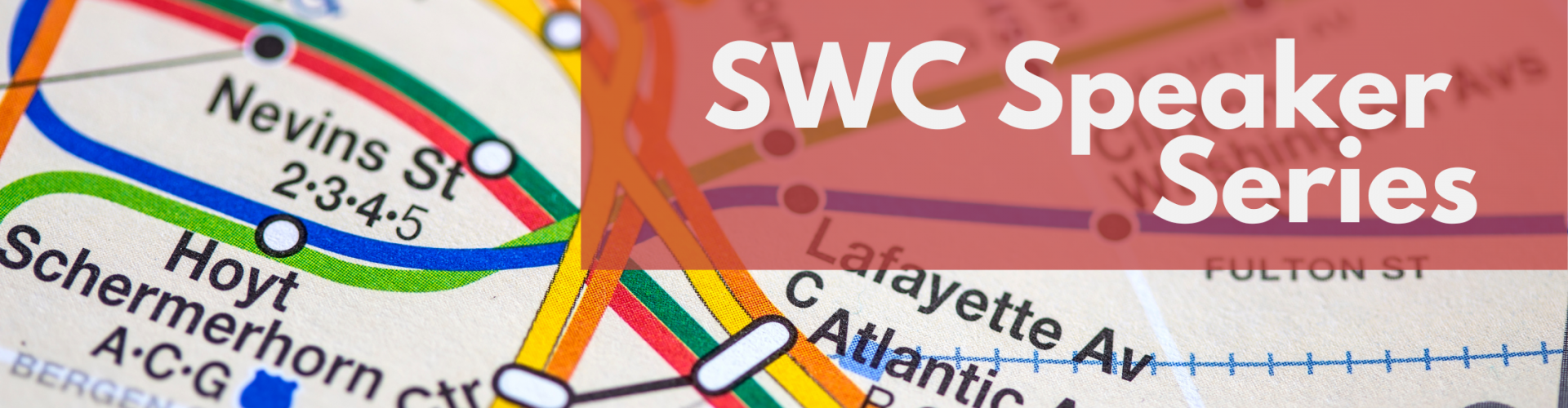
How does the brain use memories to make predictions?
An interview with Professor Loren Frank, University of California, San Francisco (UCSF), conducted by April Cashin-Garbutt
Every day we make predictions. For example, we often think about what will happen if we make certain choices. These predictions and evaluations of future events depend upon our memories and experience of the world. Professor Loren Frank recently gave a seminar at SWC where he discussed how research from his lab is helping to understand the structure of representations of past, present, and potential future. In this Q&A, he shares more about his research on representations in the hippocampus and their coordination with activity elsewhere in the brain.
How do animals use memories to make predictions about future events?
As far as we can tell, memories allow the brain to construct a map of experience – connecting relevant information. We often study this in the context of space. For example, we know that the hippocampus, which is critical for forming memories, also creates a map of space. This map is more akin to the tube map than a scale map – it shows things that are connected but without necessarily all the details.
We think that the hippocampus is linking to all the things in the world at each part of an experience. Going back to the London tube map example, the hippocampus allows you to remember that King’s Cross is where the train station is, and Tottenham Court Road is the closest station to the British Museum for instance.
The hippocampus doesn’t necessarily store information about those places, but it stores pointers to it. The visual areas may remember what those places look like, and parts of taste cortex might remember what your favourite restaurant near each of those places is like and so forth. Together this creates a kind of memory map or an idea of what’s out there in the world, how it’s connected, and what each thing is associated with.
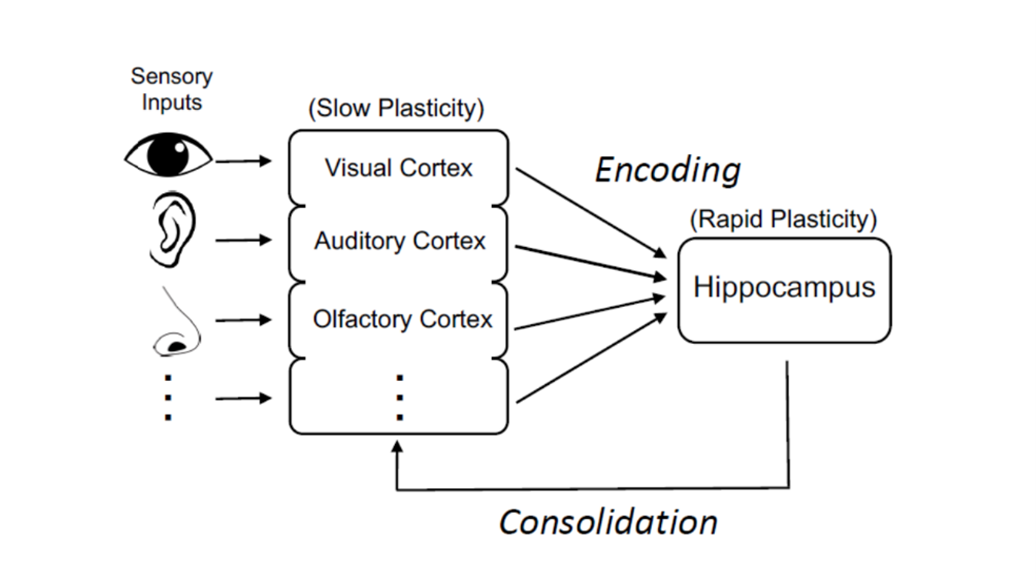
A model of information flow underlying memory (and mental simulation)
If you have this memory map, then you can start to use it to make predictions. For example, if I get off the tube at Kings Cross and start walking upstairs, I can start to make predictions about what I’m going to see. For example, you remember that your favourite coffee shop is usually on the left but if you come above ground and see it is on the right, you know you’ve taken the wrong exit.
You can also use the memory map to make predictions and evaluations. For example, if you’re hungry, you might know what restaurants are in the area and then you can think about what food you would like and make predictions about how happy you are going to be with each of the potential places. The idea is that the memories provide you with this sort of scaffolding to make predictions about what will happen if you choose different things.
How do animals know which information is useful to extract?
The brain comes with a lot of heuristics. For example, trying to get animals to pay attention to a light or an auditory cue takes an unbelievably long period of time. It can take weeks of training for an animal to learn to go left when they hear a high tone and right for a low tone for instance, because it is an incredibly unnatural thing for them to do.
On the other hand, if you give an animal a task such as foraging, where they get different probability of reward in different places, they are extremely good at sampling the possibilities and figuring out which one is the best. This is because working out the best food location is naturally a very salient thing to them.
The way I think about it is that there is stuff that evolution built in. Then there are also many things that we can layer on-top in humans, thanks to our ability to learn about what matters. This can change the way we filter information. Animals can do this too, for instance they can learn that they need to pay attention to lights in one task and then once they know this is relevant, they can pay attention in future tasks.
How long do animals store this information for and can it be overridden?
They can certainly store this information for substantial fractions of their lifetime. For example, if you give them a fear event, they will have some memory of that for a long time afterwards – certainly at least a month or so, possibly a lot longer. For example if you raised an animal in a particular burrow and then you took it out and brought it back six months, or even a year, later, it would probably find its way around much more quickly than a naive animal.
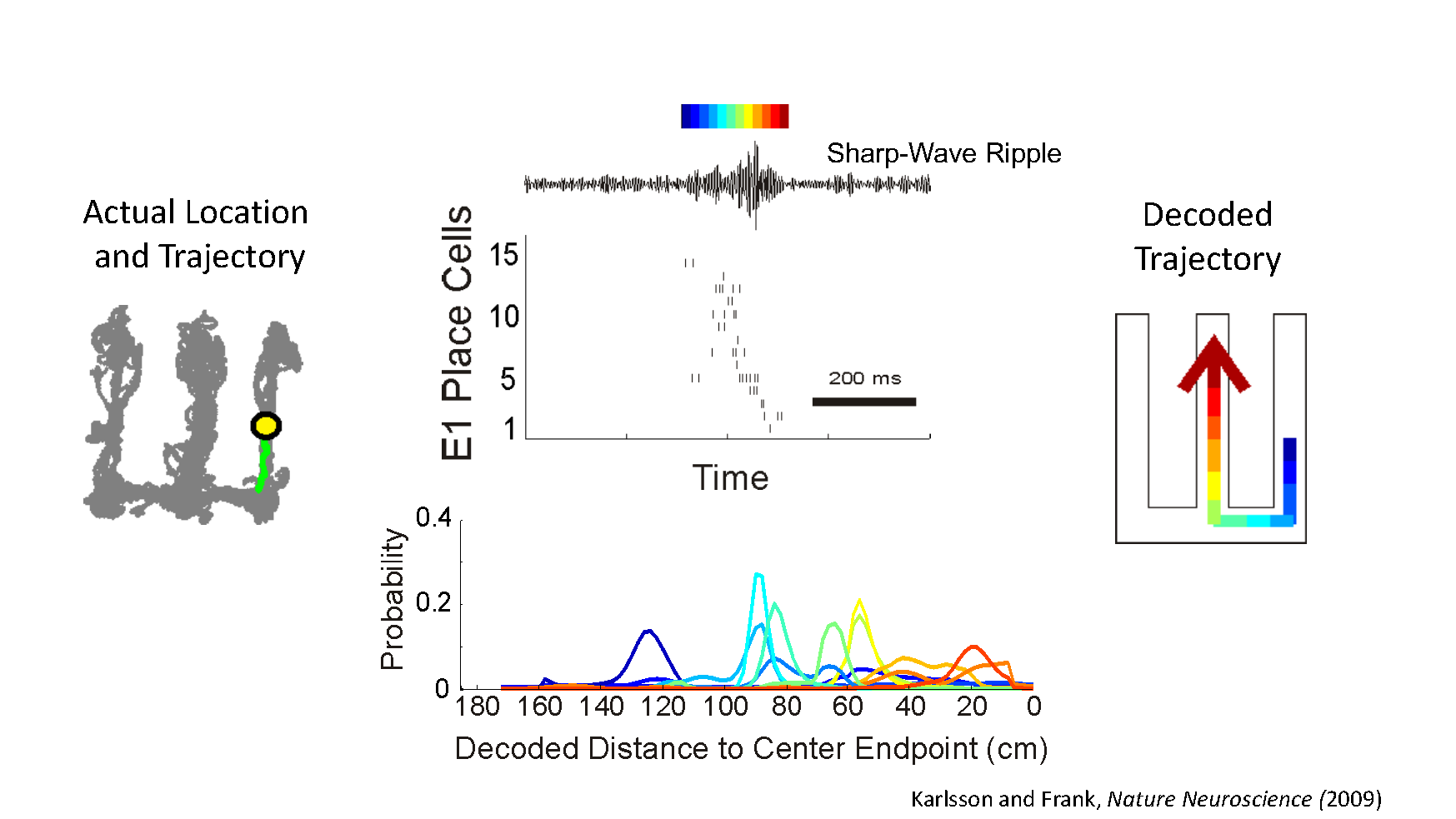
Representations of past and possible future paths
How is your research exploring the structure of representations of past, present and potential future?
One of the challenges with studying things that aren’t externally visible, is knowing when an animal is thinking about the past or mentally exploring the future. We need an anchor point in our studies. In our case, we start with the actual present in the hippocampus, which has been studied for over fifty years.
Most of the time, the hippocampus seems to be engaged in representing current experience, so that then provides a jumping off point, because we can ask when does it look like that isn’t accurate? Are there periods of time where suddenly we don’t understand what’s going on in the hippocampus because our model of it as encoding current experience doesn’t seem to accurately describe what’s going on?
Then we can jump from there to a secondary question and say if we know that’s the case, and if we know about the animal’s past experiences, can we interpret this confusing pattern of brain activity in terms of something that happened before? The easiest way to see this are in these events called replay events that happen during sharp-wave ripples (SWRs) and there you can see what look like time-compressed sequences, which can quite beautifully recapitulate something the animal did before. These usually happen when the animal is still, so it is clear that the animal is not having a sequential, real-world experience, it’s having a sequential mind experience.
It turns out there are also times when the animal is moving around where the activity doesn’t seem to correspond exactly to the animal’s current location. As the animal moves, there is a rhythm in the hippocampus that goes up and down eight times a second. It turns out if you look really closely, what you see if that the early parts of each phase of that rhythm seem to correspond more closely to what’s really going on, and then the later parts can, but don’t always, correspond to something that you might think of as what’s coming up.
You can’t know what’s coming up, unless you’ve done something before. If you are completely naïve to a new space, you can’t possibly know what’s around the corner, but once you know, as you walk up towards the corner you can start imagining or representing what might be there. This is very much what the hippocampus seems to be doing during movement.
We found that it even goes beyond that. Sometimes in the later part of each cycle, the hippocampus looks like it can jump to a faraway place. It is although the brain briefly considers something that is nowhere near where the animal is right now and then it hops back. We are all familiar with this – you can be walking down the street and you can briefly think ‘I left my watch on my bedside table’ and then you go back on with your day. And so we are seeing echoes of what might be neural substrates for that type of thing. This is all grounded in a good understanding of place cells and what the system does most of the time, and that becomes an anchor point for understanding what it could do the rest of the time.
How much is known about the way hippocampal representations are coordinated with activity elsewhere in the brain?
My lab and multiple other labs have studied SWR replay events a lot and we know there is actually quite profound coordination everywhere we look in a rodent brain. The clearest work on this was a study by Nikos Logothetis from 2012, who was in Tübingen at the time, and they did fMRI in primates where they recorded events in the hippocampus and looked at blood flow changes across the brain. They found these events were associated with blood oxygenation changes, indicative of activity, everywhere in the brain. So it looks like this was a brain-wide event.
When we went in and looked, for example in the prefrontal cortex, we found that there’s quite strong coordination between the set of hippocampal cells that are active that can predict which prefrontal cells are going to be active, as though they are carrying some sort of joint information. We found this was even true in primary auditory cortex. The current idea is that we think these replay events are memories that are reinforcing and updating events.
If you think about these memories as where you are, connected to all the things in the world that you experience, then it makes sense that you might need to turn back on all that stuff to help link it back together. For instance, what sounds did I hear, what task was I doing, what did it smell like? You might want all that information to come back on together. And so, that’s the hypothesis why the brain might organise these brain-wide events.
In the context of theta, which happens when the animal is running, we actually know a lot less. We know that many parts of the rodent brain seem to oscillate at 8Hz and also that rodents run at 8Hz in terms of when their feet hit the ground. Our work suggests that there are times when there is coordination from the sensory motor periphery all the way to the hippocampus, implying coordination everywhere in between and suggesting that the whole system is working together and processing information of different sorts, maybe on different phases of this cycle.
We know that in pre-frontal cortex, many cells are modulated by theta and we know that we can see activity there that is different sometimes on the early phases versus the late. This relates to what the hippocampus is doing and so there are hints that it may be some brain-wide thing as the SWRs, but we really don’t understand it yet.
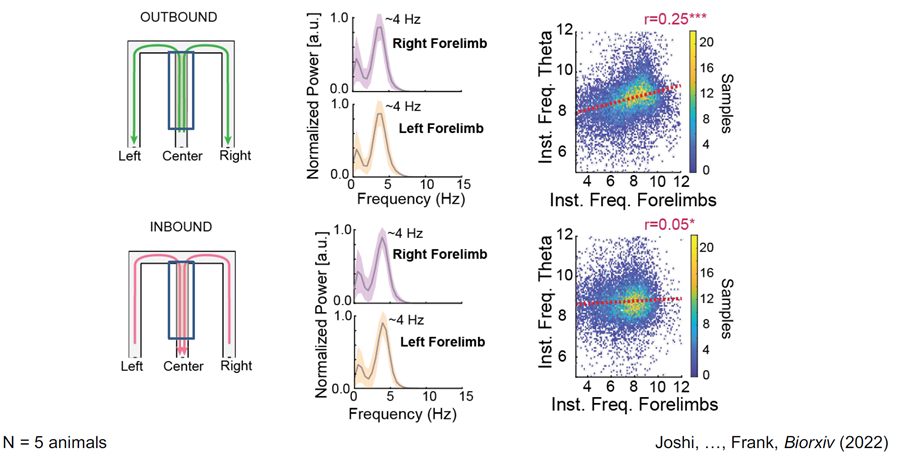
Task-phase specific coupling of hippocampal rhythms and stepping
What are the key implications of your research?
Firstly, it is possible to start talking about things like memory and imagination without having everyone laugh! I think that’s a nice state to have gotten to in science, where we can start to go after these things that are really quite fundamental to our personal experience in hopefully a rigorous scientific way. To me, that is very exciting and very philosophically satisfying.
The other implication is that we have figured out that there are times when the hippocampus is engaged in representing something other than right now. If the rest of the brain is engaged and following along, other areas that people have studied, for example visual responses, may also be thinking about the past or the future for some fraction of time, and so the current interpretation of the activity as related to the present, which is what most of neuroscience is about, may be off.
I think this is actually really exciting, because it’s been so hard to figure out what’s going on, and things seem so noisy and variable in lots of places. My own bias is that if we actually understand what is being thought about at each moment in time, then suddenly lots of things will make sense that don’t make sense now. Lots of this variability will turn into signal for possibilities, or past experiences, or predictions, rather than noise related to current experience, which is how it’s often interpreted. From my perspective, this is a fun challenge for the field if it turns out we’re right. We don’t know yet, but it is hard for me to imagine this not being true, given how much of time the brain can spend thinking about the past and future.
The final implication is that our research may help us to understand coordination and how the brain can do so many different things in so many different ways. For example, when you’re walking to a local shop, you can be thinking about lots of different things along the way. Your brain is still processing sensory information as you walk, but somehow you’ve switched this other part of your brain to be doing something completely different. Essentially your actions are automatic at that point. However, when you first move to a new area you have to actively navigate and so you probably use all these brain systems to figure out where things are and so on. Once you’re familiar with the area, you can turn these brain systems off and they don’t govern your behaviour anymore.
This profound flexibility in terms of how many different parts of the brain actually influence behaviour at different times is really mysterious, because it is all connected. You can get from anywhere in the brain to almost anywhere else quite easily. If this were a static structure, it would mean everything would always be talking with everything else and so to me it helps militate this need to understand how you prioritise some channels over others.
How do you couple the hippocampus to locomotion during times when the animal might need to think about where it is going, and how that relates to where it is and future possibilities? Then how do you completely decouple those when you don’t need to anymore? I think the mechanisms of coupling and the subtleties of that couple will also turn out to be important in terms of understanding how the brain is so flexible.
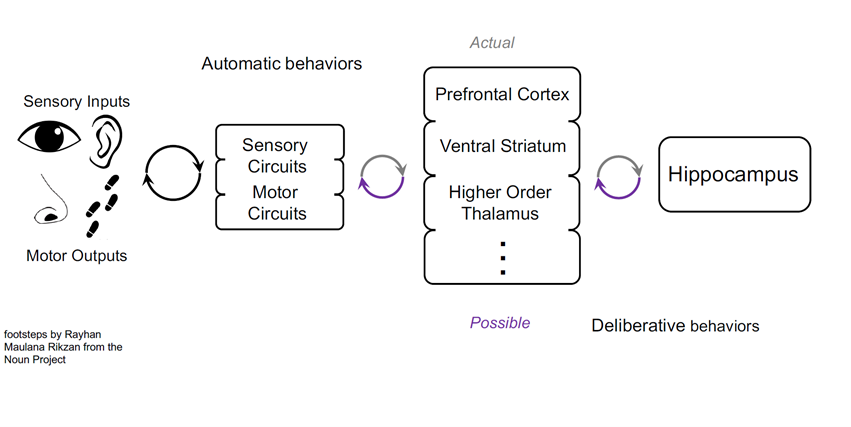
Hypothesis: Dynamic coupling allows dynamic engagement
How are you collaborating with Lawrence Berkeley, Lawrence Livermore National Laboratories and Rice University to develop new technologies to understand how the brain works and how to fix it when it is not working properly?
We’re mainly working together on two things. Firstly, flexible electrodes for long-term recording from the brain. One of the issues is that the brain is really soft and squishy, so most electrodes, like the Neuropixels probe, are made from silicon, which basically means they’re glass. The challenge with putting glass into the brain is that glass is really sharp and stiff whereas the brain is not. If there’s any movement of the brain relative to this amazing piece of glass, then it will cause damage to the brain. In larger animals, this is even more of an issue because the brain moves inside the skull much more.
Our approach has been to develop very flexible electrodes. They are made out of polymer rather than silicon, so they are orders of magnitude more flexible. The most extreme versions are ones made by our colleagues at Rice University, which are 1-2 microns thick, so they’re 1/75th the thickness of the human hair, yet they can still register signals. What we find with these electrodes is that we can get stable recordings, where the brain doesn’t move relative to the electrode, for weeks and even months. We showed a few years ago in a paper that we can follow large numbers of cells 24/7 for a week. During this time, we can collect more or less every spike and look at how those neurons evolve over time. To my knowledge, that’s something that’s not really possible with any silicon device. The hope is that with these more flexible electrodes will work well particularly in larger animals and for longer-term recordings. This technology is actually now being used by Neuralink.
In addition to developing the electrodes, the other challenge is being able to read out the signals. There are tens of millions of neurons in the rodent brain and tens of billions of neurons in the human brain and even with the latest devices we can only measure a tiny fraction of them. With Lawrence Berkeley we’ve been working on a head stage chip, which takes the signals, amplifies them and then sends them out. And so far we’ve developed one that’s about eight times as dense as the main one that people use in the market now. The idea is that we can bond this directly to these polymer devices so that we create an integrated thing that weighs less than a gram, which will be good for 512 electrodes. Then the system we’re working on will allow us to couple up to eight of those together in rat, which will be 4096 electrodes distributed across many areas.
The reason I’m excited about this is that hopefully we will be able to start to measure all this distributed coupling that I mentioned and how and when these distributed networks are engaged. I feel like we really need to be able to measure those things to figure out what’s going on. The pandemic led to a lot of setbacks for our project, but we’re pulling it back together now and I’m hoping a year from now we’ll have our first demonstration of this.
What are your thoughts on transcranial direct current stimulation (TDCS) devices and their potential impact on memory?
It is clearly possible to send electrons through the brain and change brain activity in humans or animals. Some of the work in this front that I think is really exciting doesn’t relate to memory but instead relates to stroke recovery. A colleague of mine at UCSF, Karunesh Ganguly, has done a lot of this work. They have done stimulation across a stroke site and shown better recovery. The idea is that you’re helping all the neurons that are preserved, re-engage with the rest of the network. I think this is really exciting.
In general, it is definitely possible to find the right parameters to help the system along. TDCS doesn’t adjust neurons individually, it presumably changes how excitable they are. For example, when you have a word on the tip of your tongue but you can’t remember it, presumably that’s because there is activity there but it isn’t enough. The idea might be that if you can just turn things up a little bit and amplify all of that then the system can self-organise and get over that hurdle. In the context of disease or brain damage, turning up in this way may help overcome some of those challenges.
What’s the next piece of the puzzle your research is going to focus on?
The next step is really to test the hypotheses that we’ve generated. For example, when the animal is representing a place that’s not where it is now, could it be evaluating that and using that information to make a decision? We are going to look to see whether the hippocampus is interacting with the frontal cortical regions for example, and see whether they are engaged to evaluate an imagination. Are they taking information from the hippocampus and deciding whether that is a good or bad place to go?
We are also exploring whether all of this non-local imagination-like activity is really important for the animal to make these decisions. We know that the hippocampus is important, but we want to find out whether this non-local activity, for example when the animal is running around, is really critical to making good decisions. To do this we need to selectively remove it without removing anything else, i.e. the activity of where the animal is right now. That’s hard because these things are happening within 30-40 milliseconds of each other on each cycle of theta.
One of the things we’ve been working on are real-time systems where we can read out, with a few milliseconds lag, what the brain is doing at each moment in time. This means we might be able to disrupt the system when it is representing a possibility and leave the current activity unaffected. This kind of interfacing with the system, on its own terms, is going to be really important to dissect reality from possibility. We should be able to remove possibility and the animals should become more rigid in their thinking and less flexible etc. This is what we think we need to do to test our hypotheses and see whether we’re right about this stuff.
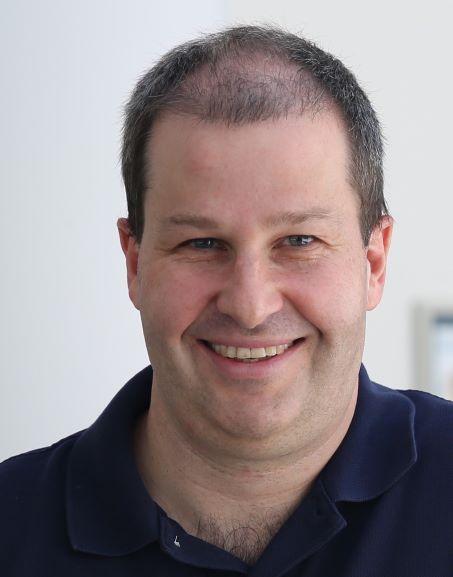
About Professor Loren Frank
Loren Frank is Howard Hughes Medical Institute Investigator and a Professor in the Department of Physiology at the University of California, San Francisco (UCSF). He is also the Director of the Kavli Institute for Fundamental Neuroscience at UCSF. He received his B.A. in Psychology and Cognitive studies from Carleton College, his Ph.D. in Systems Neuroscience and Computation from M.I.T. and did post-doctoral research at Massachusetts General Hospital and Harvard University. His laboratory uses a combination of techniques to study the neural bases of learning, memory and decision-making. In particular, his work focuses on the hippocampus and related structures, brain areas critical for forming and retrieving memories for the events of daily life. He also works in close collaboration with colleagues from Lawrence Berkeley and Lawrence Livermore National Laboratories to develop new technologies to understand how the brain works and how to fix it when it is not working properly. These technologies include flexible polymer electrodes that make it possible to record from large numbers of neurons for months at a time. Dr Frank has received numerous awards for his scientific discoveries and his mentoring, including fellowships from the Sloan, McKnight and Merck Foundations as well as the Society for Neuroscience Young Investigator Award, the University of Indiana Gill Young Investigator Award, the UCSF Faculty Mentoring Award, and the College Mentors for Kids Inspire Award.